Revolutionizing Energy Storage with Cryogenic Technology
- Hüsnü Tolga Eyyuboğlu
- Feb 10
- 11 min read
Updated: Mar 5
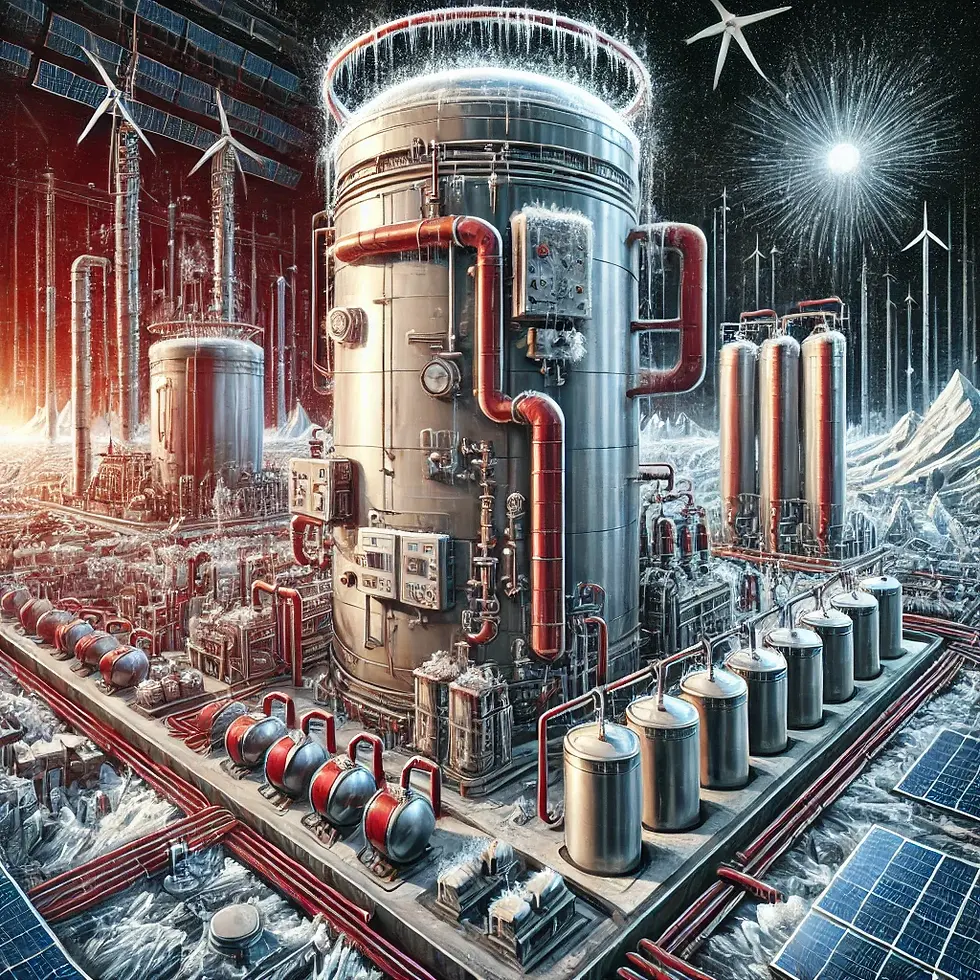
Can ultra-low temperatures solve the energy crisis? Cryogenic technology is proving that energy storage isn't just about batteries—it's about freezing power for the future. By leveraging ultra-low temperatures to store energy in innovative ways, cryogenic technology offers solutions to some of the most pressing challenges in renewable energy and industrial processes. As energy systems transition to accommodate more renewable sources, the need for reliable, scalable, and efficient storage solutions has never been greater.
This blog article explores cryogenic technology and its applications in energy storage, and their transformative potential for a sustainable future across three key areas. This is also a series of blogs. More detailed blogs/articles of energy storage systems are available in our blog. You can follow the links.
What is Cryogenic Technology?
Cryogenic technology involves the study and application of extremely low temperatures, typically below −150°C (−238°F). This field enables the manipulation and storage of materials in a highly stable state, making it ideal for energy storage applications. By cooling gases like nitrogen, oxygen, and hydrogen to their liquid states, cryogenic systems can achieve unparalleled energy density and efficiency.
Key Features of Cryogenic Technology:
Temperature Control: Maintains materials at ultra-low temperatures to preserve their physical properties.
Energy Efficiency: Reduces energy loss by minimizing thermal activity.
Scalability: Suitable for large-scale industrial and grid applications.
Cryogenic Energy Storage
Liquid Air Energy Storage (LAES) is an emerging technology designed to address the challenges of large-scale, long-duration energy storage, especially for renewable energy sources. By leveraging the thermodynamic properties of air, LAES systems store excess energy during periods of low demand and release it when needed, thereby enhancing grid stability and reliability.
The LAES process consists of three key stages:
Air Intake & Liquefaction: Ambient air is drawn in and purified to remove contaminants such as CO₂ and water vapor. The purified air is then cooled to approximately -196°C (-320.8°F) using a combination of the Linde-Hampson Cycle and Joule-Thomson expansion, significantly reducing its volume for efficient storage.
Cryogenic Storage: The liquefied air is stored in insulated, low-pressure tanks at near-atmospheric pressure, minimizing thermal ingress and energy losses. Advanced vacuum-insulated containers help maintain ultra-low temperatures with minimal boil-off.
Energy Recovery & Expansion: During peak demand, the liquid air is pumped to high pressure, reheated using ambient or waste heat, and expanded through turbines to generate electricity. Efficiency can be further improved by recovering waste heat from industrial sources or integrating thermal energy storage systems.
This innovative approach enables large-scale, long-duration energy storage, making LAES a flexible and sustainable solution for grid stabilization and renewable energy integration.
One of the main advantages of LAES technology is its high energy density, allowing significant energy storage in a relatively small volume. Additionally, LAES systems have long lifespans, often exceeding 30 years, thanks to the use of durable industrial components. Another key benefit is geographical flexibility; unlike other large-scale energy storage solutions such as pumped hydro, LAES plants can be constructed without geographical constraints, making them suitable for urban and industrial locations. Furthermore, LAES systems utilize air as the storage medium, eliminating hazardous materials and contributing to environmental sustainability.
Several case studies demonstrate the feasibility and scalability of LAES technology. Highview Power, a UK-based company, has pioneered the deployment of LAES systems with a 5 MW/15 MWh pilot plant in Greater Manchester. The company is also constructing a 50 MW/250 MWh commercial facility in Carrington, expected to be operational by 2026. These projects highlight the technology's potential to scale from pilot stages to full commercialization. The current Technology Readiness Level (TRL) of LAES is estimated at 8–9, indicating it is in the final stages of development and deployment in operational environments.
Hydrogen is increasingly recognized as a pivotal element in the transition to sustainable energy systems, serving as a clean energy carrier with applications spanning transportation, power generation, and various industrial processes. However, storing Hydrogen efficiently remains a major challenge due to its low volumetric energy density in gaseous form. Liquid Hydrogen, which involves liquefying Hydrogen at extremely low temperatures of approximately -252.87 °C (-423.17 °F), offers a promising solution by significantly reducing its volume for efficient storage and transportation.
Maintaining Hydrogen in its liquid state requires specialized cryogenic storage systems equipped with multi-layer insulation (MLI) and vacuum jackets to minimize heat ingress and reduce boil-off losses. Stainless steel and aluminum alloys are commonly used materials for these tanks due to their ability to withstand extreme temperatures and maintain structural integrity.
Liquid Hydrogen offers several significant advantages. High energy density allows for the storage of large amounts of energy in a compact volume, making it suitable for applications where weight and space are critical, such as in aerospace and automotive sectors. The lightweight nature of Liquid Hydrogen storage further enhances its potential in transportation applications, including hydrogen-powered vehicles, buses, and aircraft. Additionally, Hydrogen serves as a clean energy carrier, producing only water vapor upon combustion, which contributes to reducing greenhouse gas emissions, especially when produced from renewable energy sources.
Despite its potential, Liquid Hydrogen faces several challenges. One of the most significant is boil-off losses, where some Liquid Hydrogen inevitably evaporates due to heat ingress. Managing these losses is crucial to prevent fuel wastage and ensure safety. Technologies such as Zero Boil-Off (ZBO) systems, which integrate cryocoolers to capture and reuse evaporated hydrogen, have been developed to address this issue. Another key challenge is material selection, as Hydrogen can cause embrittlement in some materials at cryogenic temperatures. Ongoing research focuses on using specialty resins and carbon fiber composites to improve the durability and safety of storage vessels. Additionally, cost considerations play a major role, as the energy-intensive liquefaction process consumes approximately 10–13 kWh per kilogram of Hydrogen, increasing the overall cost.
Compressed Hydrogen (CGH2): Requires 4-6 kWh per kg at 700 bar, making it less energy-intensive than liquefaction but requiring heavier, high-pressure tanks.
Solid-State Hydrogen Storage (Metal Hydrides/LOHCs): Provides low-pressure alternatives, but these methods increase system complexity and often require thermal management.
Advancements in liquefaction efficiency and economies of scale are essential to reduce costs and improve economic viability.
The Technology Readiness Level (TRL) of Liquid Hydrogen varies depending on the application, with aviation-related systems currently at TRL 3 (experimental proof of concept). The Aerospace Technology Institute’s FlyZero project aims to achieve TRL 6 by 2026, which would involve prototype demonstrations in relevant environments. (While this is an exciting development, the feasibility of TRL 6 depends on advancements in material science (e.g., hydrogen embrittlement solutions) and cost-effective cryocooler technology.) Ongoing research focuses on improving materials, enhancing system integration, and optimizing boil-off mitigation strategies.
When compared to other Hydrogen storage methods, Cryogenic Storage presents distinct advantages and challenges. Compressed Hydrogen Gas, stored at high pressures of up to 700 bar, offers rapid refueling capabilities but requires costly, robust tanks. Metal Hydrides allow for low-pressure storage but have lower energy densities and slower release rates. Liquid Organic Hydrogen Carriers (LOHCs) enable storage in a liquid state at ambient temperatures but require additional energy for Hydrogen extraction. Ammonia, a Hydrogen Carrier with higher energy density, offers an alternative but necessitates cracking technologies to release Hydrogen, which adds complexity and cost.
Liquefied Natural Gas (LNG) plays a crucial role in the global energy landscape by providing a versatile and efficient solution for storing and transporting natural gas. LNG is produced by cooling natural gas to approximately -162 °C (-260 °F), which reduces its volume by 600 times, making it easier to store and transport over long distances.
Once liquefied, LNG is transported via specialized carriers equipped with insulated tanks that maintain cryogenic conditions. Upon arrival at regasification terminals, LNG is reheated and converted back into its gaseous state before being injected into pipelines for distribution to end-users. As of 2024, there are over 600 LNG carriers operating globally, reflecting the increasing demand for LNG transportation. Compared to pipeline gas, LNG offers greater flexibility in terms of supply chain management and global reach, whereas pipelines require significant infrastructure investments and are limited by geographical constraints.
LNG is widely used across various sectors, including power generation, transportation, and industrial processes. In power generation, LNG is considered a cleaner alternative to coal and oil, emitting 50-60% less CO₂, thereby contributing to global decarbonization efforts. In the transportation sector, LNG is increasingly adopted as a fuel for heavy-duty trucks and marine vessels to comply with stringent International Maritime Organization (IMO) sulfur emission regulations. In industrial applications, LNG serves as an energy-efficient and cost-effective feedstock for industries such as chemicals, manufacturing, and fertilizers, offering a reliable alternative to traditional fossil fuels.
Despite its advantages, LNG infrastructure requires substantial investments. The capital expenditure (CapEx) for an LNG terminal can range from $500 million to $5 billion, depending on its capacity and location. For example, the Guangdong Energy Group's LNG terminal in Huizhou, China, was developed at an estimated cost of $1 billion, featuring a handling capacity of 6.1 million tons annually and three storage tanks, each with a capacity of 200,000 cubic meters. Operational expenditure (OpEx) also remains a challenge, with the liquefaction process, involving multiple cooling stages using refrigerants in a cascade cycle, consuming up to 15% of the total energy content, which impacts the overall efficiency and cost-effectiveness of the technology.
The Technology Readiness Level (TRL) of LNG infrastructure varies depending on the specific technology. Traditional liquefaction and regasification processes are at TRL 9, meaning they are fully commercially deployed. Small-scale LNG projects, designed to serve niche markets and remote areas, are at TRL 7-8, indicating they are in the late development stages with ongoing pilot demonstrations. Floating LNG (FLNG) facilities, such as Shell’s Prelude FLNG, have also reached TRL 8-9, proving their feasibility and scalability for offshore gas production and processing.
Benefits of Cryogenic Energy Storage
Cryogenic energy storage (CES) offers several advantages over conventional energy storage methods, making it a compelling solution for modern energy challenges. One of the most significant benefits is sustainability. Cryogenic systems utilize renewable energy sources, such as wind and solar, to power the liquefaction and storage processes, thereby reducing the overall environmental footprint. Unlike battery storage, which involves mining-intensive materials such as lithium and cobalt, cryogenic storage systems rely on abundant atmospheric gases like air or nitrogen. This makes CES a cleaner alternative with minimal resource depletion. Furthermore, by enabling the storage and efficient utilization of excess renewable energy, cryogenic systems help decrease dependence on fossil fuels, supporting global carbon reduction targets. For example, Highview Power’s liquid air energy storage (LAES) facility in the UK integrates wind power to store energy and supply it to the grid during peak demand, reducing the need for natural gas-based power generation.
Another critical advantage of cryogenic storage is scalability. Cryogenic energy storage systems can be designed to suit a wide range of applications, from small-scale industrial facilities to large utility-scale grid support projects. Unlike traditional energy storage methods such as pumped hydro, which require specific geographical features, cryogenic storage can be deployed in diverse locations without significant infrastructure modifications. The modular nature of cryogenic storage allows for incremental expansion, making it a cost-effective solution as energy demands grow. For example, the CryoBattery system developed by Highview Power can be scaled from 10 MW to 100 MW, providing flexibility to accommodate varying power needs across urban and rural areas.
Safety and reliability are also key strengths of cryogenic energy storage. CES systems store energy in the form of liquefied gases at ultra-low temperatures, which inherently reduces the risk of combustion or hazardous chemical reactions compared to other storage methods, such as lithium-ion batteries, which are prone to thermal runaway and fire hazards. Additionally, cryogenic storage systems have long operational lifespans, with minimal performance degradation over time, ensuring consistent energy delivery. Unlike chemical batteries, which degrade after several charge-discharge cycles, cryogenic systems can operate efficiently for over 30 years with proper maintenance. For instance, the LAES facility in Manchester, UK, has demonstrated stable performance with low maintenance requirements, positioning cryogenic storage as a highly reliable option for grid stability and backup power.
In comparison to other energy storage technologies, cryogenic systems offer distinct benefits such as higher storage capacity, longer discharge durations, and fewer geographical constraints. While lithium-ion batteries provide fast response times, they are limited in duration and energy capacity. In contrast, cryogenic storage can provide long-duration energy storage (from several hours to days), making it ideal for balancing seasonal variations in renewable energy production. Additionally, CES systems do not face the environmental disposal issues associated with chemical battery waste, further reinforcing their sustainability advantage.
Challenges and Future Directions
Cryogenic energy storage (CES) offers numerous advantages; however, several challenges must be addressed to ensure its widespread adoption and long-term viability. One of the most significant hurdles is the high initial cost associated with cryogenic storage infrastructure. Setting up a cryogenic storage facility requires substantial capital investment in specialized equipment, such as cryogenic tanks, compressors, and heat exchangers, which are more expensive compared to traditional energy storage solutions like lithium-ion batteries and pumped hydro. For example, the Highview Power 50 MW liquid air energy storage (LAES) plant in the UK has an estimated capital cost of over £300 million, making it a challenging investment for utilities and industries with budget constraints. However, the long operational lifespan of CES (up to 30 years or more) can offset these high initial costs by providing long-term returns with minimal degradation. Additionally, advancements in materials, insulation technologies, and economies of scale are expected to reduce installation and operational expenses. Government incentives and public-private partnerships are also playing a crucial role in accelerating cost reductions by funding pilot projects and offering tax credits for energy storage technologies.
Another major challenge is the high energy intensity of the liquefaction process. Liquefying air or hydrogen requires cooling it to extremely low temperatures, such as -196 °C (-320 °F) for air and -252 °C (-423 °F) for hydrogen, which consumes a significant amount of energy—typically 10-13 kWh per kilogram of hydrogen. This energy input can negate some of the efficiency benefits of cryogenic storage if not managed effectively. To improve efficiency, innovations in renewable energy integration, such as using surplus wind or solar power for liquefaction, are being explored. Additionally, the implementation of waste heat recovery systems from industrial processes and power plants can help reclaim energy lost during liquefaction, improving overall energy efficiency. Companies like Air Products and Linde are developing advanced liquefaction techniques that incorporate heat exchangers and energy recapture technologies to minimize energy consumption and enhance performance. When compared to other energy storage technologies such as pumped hydro (which has an efficiency of around 70-80%), cryogenic energy storage currently lags in terms of round-trip efficiency but offers advantages in flexibility and scalability.
As breakthroughs in insulation, liquefaction efficiency, and hybrid energy systems continue, cryogenic technology is on track to become a key player in the global energy transition. Handling cryogenic liquids involves maintaining extremely low temperatures, which can pose risks such as frostbite, equipment failure, and the potential for leaks leading to hazardous situations like oxygen displacement in confined areas. Strict compliance with safety regulations, such as those set by the Occupational Safety and Health Administration (OSHA) and the European Industrial Gases Association (EIGA), is mandatory to ensure the safe operation of cryogenic storage systems. The implementation of advanced safety technologies, including automated leak detection, pressure relief valves, and redundant containment systems, is essential to mitigate risks. Additionally, continuous training and education of personnel handling cryogenic systems are necessary to prevent accidents and ensure operational safety. Compared to other storage methods like battery systems, which are prone to thermal runaway and fire hazards, cryogenic storage offers a safer long-term alternative if proper safety measures are adhered to.
Looking ahead, the future of cryogenic energy storage is promising, with several areas of technological advancement and market opportunities on the horizon. One key area of research focuses on enhancing the round-trip efficiency of CES by integrating advanced insulation materials, superconducting technologies, and energy recovery systems. Companies and research institutions are exploring hybrid energy storage systems that combine CES with batteries to optimize grid balancing and response times. The transportation sector is another promising avenue, with liquid hydrogen storage emerging as a critical component in the transition to zero-emission fuel for aviation and heavy-duty trucking. Airbus and Boeing are currently researching cryogenic hydrogen storage systems for long-haul flights, with the potential to replace conventional jet fuel in the coming decades.
Additionally, the expansion of cryogenic storage in developing markets offers significant growth potential. As countries with growing energy demands, such as India and China, seek sustainable storage solutions, cryogenic systems can provide reliable energy storage without geographical limitations. Unlike pumped hydro storage, which requires specific terrain conditions, cryogenic storage facilities can be built in urban and industrial areas, offering greater deployment flexibility.
Cryogenic energy storage (CES) presents a transformative opportunity to address the growing global need for reliable, scalable, and sustainable energy solutions. As the world transitions toward renewable energy sources, the inherent intermittency of solar and wind power underscores the importance of advanced storage technologies. Cryogenic storage systems, such as Liquid Air Energy Storage (LAES), Cryogenic Hydrogen Storage, and Liquefied Natural Gas (LNG), offer unique advantages, including high energy density, long-duration storage capabilities, and minimal geographic constraints. These systems provide a viable alternative to conventional storage solutions, such as batteries and pumped hydro, by offering longer operational lifespans, lower environmental impact, and enhanced grid stability.
Despite their promise, challenges remain, particularly in terms of high initial capital costs, energy-intensive liquefaction processes, and regulatory complexities surrounding the handling of cryogenic materials. However, ongoing advancements in energy efficiency, waste heat utilization, and material innovations are expected to drive down costs and improve overall system performance. Government incentives, private sector investments, and increased adoption across industries will further accelerate the commercialization and deployment of cryogenic storage solutions.
Looking ahead, cryogenic energy storage is poised to play a critical role in decarbonizing the energy sector, enabling the large-scale integration of renewables, supporting cleaner transportation fuels, and enhancing industrial energy efficiency. With continued technological improvements and supportive policies, CES can become a cornerstone of a sustainable and resilient energy infrastructure, meeting the demands of future generations.
Comments